Orbital Mechanics
The mission to Epsilon Eridani is arguably a greater
challenge than space missions executed in the past. The interstellar mission will
require the spacecraft to travel 10.5 light years to the destination, and once
there, transmit a signal carrying images of bodies in the Epsilon Eridani system.
One concern in the mission is the operational lifetime of the equipment on
board. Long travel times may lead to deterioration in the performance of the subsystems and various instruments.
Thus, it is desirable to develop a
trajectory which minimizes the travel time to Epsilon Eridani given current
technologies. The trajectory will employ several gravity assists which will
accelerate the spacecraft out of the solar system. Once the spacecraft has
reached the bounds of the solar system, the ion drive will be activated to
further accelerate the spacecraft beyond the solar system into the Epsilon
Eridani star system. At approximately half of the distance to the Epsilon
Eridani star system, the spacecraft will rotate 180 degrees and decelerate. The location of the Epsilon Eridani relative to the Solar system is shown in Figure 1 while a comparison of the project size of the Epsilon Eridani relative to the Solar system is shown in Figure 2
The spacecraft will be launched into a geostationary
transfer orbit (GTO) using the Falcon 9 Heavy. The GTO will have an apogee
altitude of 37,500 km and a perigee of 185 km. From GTO two options were
considered to accelerate the spacecraft out of the earth solar system. These
were: 1) utilizing only the propulsion system, accelerate the spacecraft to the
edge of the solar system (i.e. a direct trajectory) and 2) employ a set of
gravity assists maneuvers to accelerate the spacecraft to the edge of the solar
system. The first option, while it appears easier operationally, possesses a
number of limitations, the main one of which stems from the trade-off between
fuel and payload. The second option, gravity assists, reduces the required
fuel to get to the outer edges of the solar system. However, utilizing gravity
assists involves more complex trajectory design and analysis. Gravity assists are
enabled by an exchange of energy between the planet and the spacecraft, and
provides “free” energy to the spacecraft (i.e. no thrust employed). The
spacecraft utilizes the gravitation pull of the planet to increase its angular
momentum, and in doing so, reduces the angular momentum of the planet. Thus,
the velocity of the spacecraft as it escapes orbit around the planet (Vout) is
greater than the velocity of the spacecraft as it enters orbit around the
planets (Vin). For the given mission, the spacecraft will use a set of three
gravity assists, Venus-Earth-Jupiter, to escape the solar system. This trajectory
developed using a trajectory analysis tool currently in development by Demyan
Lantukh in the Space System Design Lab at Georgia Tech. Figure 4 provides the survey of all trajectories considered, with each trajectory represented by a
yellow dot on the graph. The trajectory selected was the one which provided the
greatest spacecraft velocity, that is, the Venus-Earth-Jupiter gravity assist
trajectory. The accumulated time indicates the time to the edge of the solar
system which is approximately 21 years. The launch data occurs in 2018 as indicated by the time axis. As a result, the
heliocentric escape velocity is 16km/s.
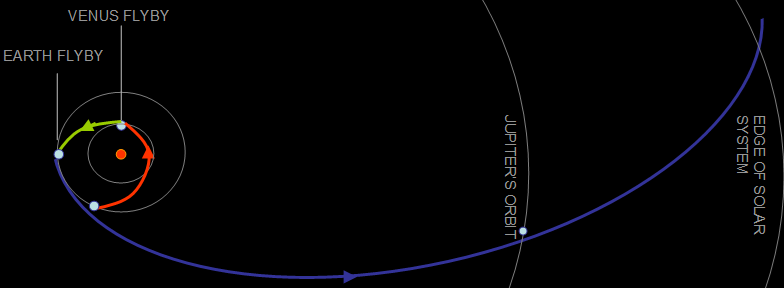
Figure 3. Illustration of Spacecraft Trajectory

Figure 4. Possible Trajectories of the Spacecraft
On exiting the solar system, the ion engines are activated. Given
a specific impulse of approximately 2326s, and a constant thrust of
approximately 246mN applied for around 4500 years, the spacecraft is capable
of reaching velocities of up to 360 km/s and reaching the intended destination
in about 10,000 years as shown in the plot below. Upon entering the edge of Epsilon
Eridani star system, the spacecraft rotates 180 degrees, ignites the ion engines
thereby applying a constant thrust of 246mN and slowing the spacecraft to around
330 km/s. This reduction in speed enables images of the planet to be captured
and transmitted back to earth.
Launch Vehicle
The primary driver in the selection of the launch vehicle is
mass of the spacecraft and the desired earth orbit in which the spacecraft
will be placed before embarking on its interstellar trajectory. The desired
earth orbit will be a geostationary transfer orbit (GTO). Thus a launch vehicle
selected must have the capability to lift a payload equivalent to the mass of
the spacecraft to this orbit. Given that the objective of the interstellar mission will
be to transmit images from the Epsilon Eridani star system, the primary systems
which will drive the mass of the spacecraft are the communications and power sub-systems.
The projected mass of the power
system required to operate the communication system and transmit images a
distance of 10.5 light years is approximately 3000kg. Using parametric mass
estimating relationship (Wertz and Larson 2007), the mass of the spacecraft is
expected to be around 19,000kg.
Based on the projected mass of the
spacecraft and the desired orbit, a Falcon 9 Heavy was selected for the
mission. The Falcon 9 Heavy launch vehicle has the capability of lifting
19,500kg to GTO, or approximately 3% more capability than required. This extra
capability will provide a margin for mass growth. The large payload fairing of
the Falcon 9 Heavy will accommodate the size of the interstellar spacecraft.
The Falcon 9 fairing is 17ft (5.22m) in diameter and the
standard payload fairing door is a maximum of 2ft (0.61m). The integrity
of the spacecraft will be maintained and protected from excess vibration with
the payload fairing through the combination of various acoustic surfaces. The
Falcon 9 Heavy comprises of two stages and bears a similar configuration to the
Falcon 9. While the second stage is similar to the Falcon 9, the first stage of
the Falcon 9 Heavy consists of two additional Falcon 9 first stages acting as
liquid boosters. A single first stage of the Falcon 9 launch vehicle has nine SpaceX
Merlin engines with each engine, powered by liquid oxygen (LOX) and rocket
grade kerosene (RP‐1),
providing 125,000 lbf sea level thrust. This gives a total thrust on liftoff of
just over 1.1 million lbf per Falcon 9 first stage. After engine start, Falcon
9 Heavy is held down until all vehicle systems are verified as functioning
normally before release for liftoff. The second stage tank of Falcon 9 Heavy is
a shorter version of the first stage tank and is powered by a single Merlin
engine with an expansion ratio of 117:1 and a nominal burn time of 345 seconds.
The engine has dual redundant pyrophoric igniters to provide additional
reliability during restart.
An image of the Falcon 9 Heavy and the dimensions of the payload fairing are shown in Figure 5. Detailed characteristics of the launch vehicle are shown in Table 1.
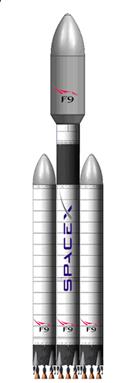 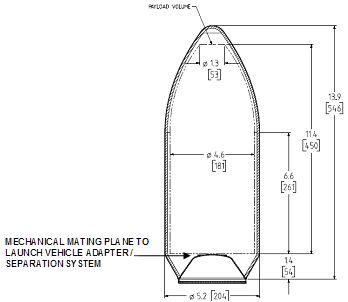
Figure 5. Image of Falcon 9 Heavy and Dimensions of Payload Fairing
Source: http://www.spacex.com/Falcon9UsersGuide_2009.pdf
Table 1. Characteristics of the Launch Vehicle
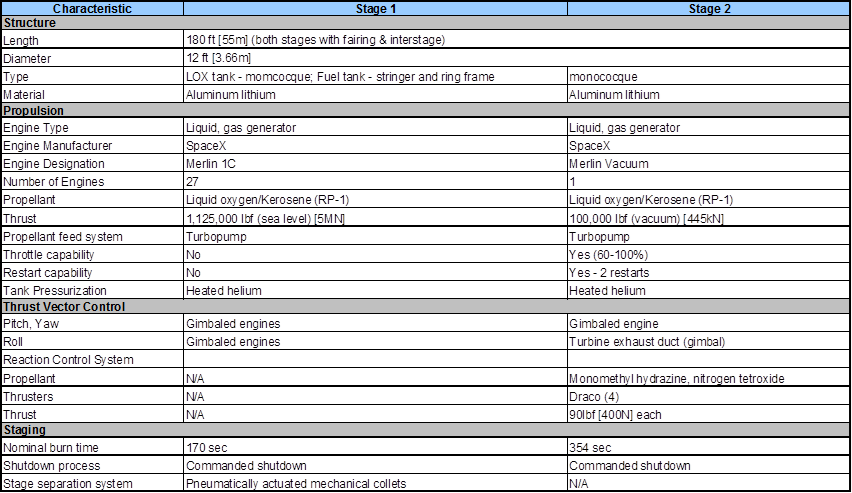
Source: http://www.spacex.com/Falcon9UsersGuide_2009.pdf
This site is © Another ECE 6390 Project
|