Propulsion System
The mission to Epsilon Eridani does not necessarily require a quick journey. However, it is clear from analyzing other subsystems that a fast journey would improve overall spacecraft performance. Long mission durations will reduce the effectiveness of a nuclear power solution and require the storage of extra fuel rods. Longer periods of time also increase the chances of political or social change on Earth, which could prevent the ground-based operations support from being produced even after launching the mission.
Gravity assist, or the slingshot effect, will need to be used to maximize the spacecraft’s velocity. An important consideration for performing the gravity assist is to ensure that the spacecraft has sufficient escape velocity for the planets it will be approaching.
Advanced
Capabilities
- Fission Rockets: The byproducts of the nuclear fission reaction are leaked into space, causing propulsion. Estimates place exhaust velocity up to 15,000 km/s, however the radioactivity involved would require substantial shielding for other spacecraft subsystems.
- Fusion Rockets: Theoretical fusion rockets could reach speeds up to 10% of the speed of light, or 30,000 km/s, based on the energy produced by the reactor. Research into nuclear fusion is promising, however current estimates place a nuclear propulsion system around the size of the space station (ref 7).
- Nuclear Pulse Propulsion: In the early 1960s, Project Orion considered the use of nuclear bombs to propel a spacecraft and showed that the concept (shown below, ref 8) was effective; weight was not a concern given the massive amount of energy in a nuclear explosion. Project Longshot, in the 1980s, formulated a 100-year trip to Alpha Centauri B, at 13,400 km/s using this technique. Unfortunately, nuclear pulse propulsion is illegal under the Partial Test Ban Treaty of 1963.
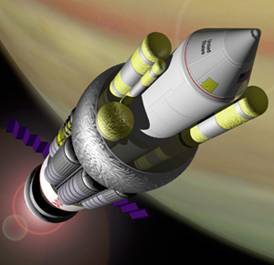

The advanced capabilities listed here could possibly support this type of mission in the future. However, a near-term mission would be limited to proven techniques such as ion propulsion, discussed here.
Mass Allocation
The spacecraft mass allocation is as follows:
Subsystem |
Component |
Mass
(kg) |
Sum
(kg) |
Communication |
Travelling Wave Tube Amplifier (TWTA) |
34 |
|
|
Dish antenna (50 meter diameter) - 1.82 kg per meter2 |
3574 |
|
Power |
SAFE-400 reactor with 4 heat exchangers - 800 kg per reactor |
800 |
|
Propulsion |
HiPEP electrostatic ion thruster (40 kW) - 11 kg / kW for a similar thruster |
443 |
|
|
Xenon fuel for ion thrusters |
4179 |
|
|
|
|
9030 |
Electronics & Structures |
Overhead @ 20% of current non-fuel mass |
970 |
|
|
Spacecraft Mass |
|
10000 |
Payload Launch Engine
A solid rocket booster launch vehicle, such as the Atlas V (ref 1), is capable of launching payloads ranging from 10 Mg to 29 Mg. The Atlas rocket itself has a launch mass of 333 Mg in its simplest configuration using just a single common core booster, which provides 3827 kN of thrust.
Insertion into LEO at 1000 meters will achieve an orbital velocity of 7349 m/s.
Payload Primary Engine
Based on the projected mission duration of many centuries, it is clear that an ion thruster solution is the best long-term option for this mission. The tradeoff in this case is time; a chemical-based propellant system will accelerate the spacecraft faster, at the cost of higher mass. A nuclear propellant system is also an option, given the nuclear reactor already onboard the spacecraft, but there is not sufficient space heritage for this approach.
Ion thrusters tend to fail when their materials degraded from extended use. In an ion thruster system, gas (typically xenon gas) is ionized and accelerated to high speeds to produce propulsion. In order to accelerate the gas, a voltage differential is developed using mesh screens; these screens can become clogged or corroded over time, leading to failure of the ion engine.
The High Power Electric Propulsion (HiPEP) ion thruster (shown below) is one possible mission candidate already in development. With a target lifetime of 10 years, the HiPEP thruster has been targeted for use on the Jupiter Icy Moons Orbiter (JIMO) mission. The thruster requires 40 kW and has a specific impulse of 9620 seconds. This specific impulse states that 1 lb (0.45 kg) of Xenon propellant will produce 1 lb (0.45 kg) of force for 9620 seconds.
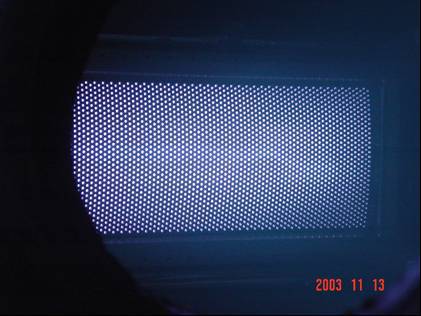
Testing performed on the HiPEP yielded the following performance results (ref 1):
Power,
kW |
Flow
Rate, mg/s |
Efficiency |
Thrust,
mN |
Specific
Impulse |
9.7 |
4.0 |
0.72 |
240 |
5970 |
15.9 |
4.9 |
0.74 |
340 |
7020 |
20.2 |
5.6 |
0.75 |
410 |
7500 |
24.4 |
5.6 |
0.76 |
460 |
8270 |
29.6 |
6.2 |
0.80 |
540 |
8900 |
34.6 |
6.6 |
0.77 |
600 |
9150 |
39.3 |
7.0 |
0.80 |
670 |
9620 |
For the Epsilon Eridani mission, the thruster will be operated around 40 kW, with a thrust of 670 milli-Newtons and a specific impulse of 9620 seconds, for the acceleration/deceleration cycles.
The mass of the ion thruster is not provided by (ref 2), however a mass can be extrapolated from the NSTAR ion thruster specification (ref 3). NSTAR has a 25.48 kg mass for 2.3 kW power, which is approximately 11 kg/kW. This implies a 443 kg mass for HiPEP.
Gravity Assist Maneuvers
The spacecraft’s speed will not be significantly affected by the ion thrusters, so gravity assist maneuvers are necessary to escape the solar system and reach Epsilon Eridani in a reasonable amount of time. The gravity assist provided by a planet can be simplified to (ref 4):
Vafter = Vbefore + 2*Vplanet
Applying the simplified model, the spacecraft orbit can be broken into the following sections. Planet velocities are found in (ref 5). This calculation requires the assumption that the planets are properly aligned to support an intricate gravity assist maneuver; in reality, intermediate points in space might need to be found to allow planets to move into position.
Gravity Assist #1: Earth to Venus (1/2 orbit)
The spacecraft velocity is 7349 m/s upon leaving orbit around the Earth. Venus has a mean orbital velocity of 35.03 km/s, so a slingshot around Venus will result in Vafter = 77409 m/s. As the spacecraft departs Venus, a small thrust is provided to prepare the spacecraft for gravity assist #2. The total time used for this assist is:

Gravity Assist #2: Venus to Raph=2.40*10^11 meters (full orbit)
The spacecraft will travel to a point Raph away from the sun, and then swing around Venus again. This orbital radius provides enough time for Venus to again move into position. As it passes by Venus, another gravity assist is provided. The result is that Vafter = 147469 m/s. As the spacecraft departs Venus, another small thrust is provided to prepare for gravity assist #3. The total time required is:

Gravity Assist #3: Venus to Jupiter (1/2 orbit)
The spacecraft will then travel to Jupiter for its final gravity assist. The orbital velocity of Jupiter is 13.06 km/s, creating a Vafter = 173589 m/s. As the spacecraft departs Jupiter, a small thrust is provided to prepare for gravity assist #4. The total time required is:

Gravity Assist #4: Jupiter to Mars (1/2 orbit)
The spacecraft will then travel to Mars for its final gravity assist. The orbital velocity of Mars is 24.13 km/s, creating a Vafter = 221849 m/s. After reaching this speed, it is unlikely that the spacecraft could be maneuvered well within the solar system for another gravity assist. At this point, the spacecraft trajectory will be slowly changed to direct it toward Epsilon Eridani. The total time required is:

The total time spent performing gravity assists is about 7 years. However, the acceleration benefit is worth the time (i.e., 221 km/s versus 7 km/s).
Voyage Time
So far, the spacecraft has only used its ion thruster to perform small maneuvers that enable the various gravity assists to take place. After reaching the final velocity of 221849 m/s, the spacecraft will be targeted at Epsilon Eridani. If a straight-line path is assumed, the total trip will take 14198 years.
Deceleration Time
The difficult part about traveling to Epsilon Eridani at any reasonable speed is the requirement that the spacecraft enter orbit around the solar system. Without carrying massive amounts of chemical propellant, this requirement is nearly impossible to meet. To help slow the spacecraft, it will be necessary to perform reverse gravity assist maneuvers around Epsilon Eridani.
Since the Epsilon Eridani system is moving away from the Sun at 15.5 km/s, a slingshot around the star will reduce the velocity of the spacecraft by 31 km/s (resulting in Vafter=190849 m/s). Provided the planets are aligned correctly, the spacecraft can then perform a slingshot around Epsilon Eridani b.
Not much is known about the orbital speed of Epsilon Eridani b. However, it can be estimated with knowledge of the mass of the star and the distance of the planet (3.4 AU):

A reverse slingshot around the planet will reduce the spacecraft velocity to Vafter=160849 m/s. This maneuver depends on the planet being within the trajectory of the spacecraft after it completes its slingshot around Epsilon Eridani.
At this point, the ion thrusters will need to be fired almost continually to form an arc and allow the spacecraft to perform another reverse gravity assist from the sun and planet. Following this assist, the spacecraft speed will be reduced to about 100000 m/s. The same maneuver needs to be performed again to reduce the spacecraft to 39000 m/s. Without additional information on the solar system and planets, this feasibility of this maneuver is questionable (e.g., the fuel requirements, availability of other planets for reverse gravity assist).
Entering orbit around Epsilon Eridani b is another area of uncertainty. The mass of the planet has been estimated to be about 1.2 times that of Jupiter. If the same conversion factor were to apply to the radius of the planet, this would yield about an 80225 km radius.
The principle mission requirement is to take a picture of the planet. If a standard 20mm lens is assumed (ref 6), then the angle of view of such a lens is 94 degrees, as shown below.
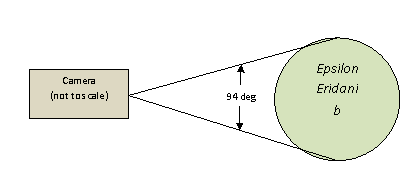
Applying a simple tangent formula, it is found that the camera’s orbit needs to be at least 116776 kilometers away from the mass center of the planet. If this information is further extrapolated, this leads to an orbital velocity as calculated (Mjup=1.8986*1027 kg):

Fortunately, this orbital velocity is very close to the current spacecraft velocity! The ion thruster must be fired to further reduce the spacecraft speed, or possibly a partial reverse gravity assist maneuver can be performed around the planet. Once the speed is at 36074 m/s or below, the orbit will be sufficient for obtaining a photograph of the entire visible side of Epsilon Eridani b.
References
(1) “Space Launchers - Atlas” Link
(2) Foster, John E., Haag, Tom, Patterson, Michael, et al. “The High Power Electric Propulsion (HiPEP) Ion Thruster”, NASA TM-2004-213194, September 2004.
(3) “GRC - NSTAR Ion Thruster”. http://www.grc.nasa.gov/WWW/ion/past/90s/nstar.htm
(4) “Double Ball Drop”. Link
(5) Windows2universe.org “Table of Planets”. Link
(6) Canon “New FD 20mm f/2.8 Lens”. Link
(7) “Space Power - NASA Science”. http://science.nasa.gov/science-news/science-at-nasa/2002/03sept_spacepower/
(8) “Eight high-tech ways to propel a spaceship | COSMOS magazine”. http://www.cosmosmagazine.com/news/2406/8-deep-space-solutions-to-infinity-and-beyond
(9) “Rocket Science :: Bussard Ramjet”. http://www.bisbos.com/rocketscience/spacecraft/bussardramjet/bussard4.html